Correlation between NADPH oxidase-mediated oxidative stress and dysfunction of endothelial progenitor cell in hyperlipidemic patients
Article information
Abstract
Background/Aims
NADPH (nicotinamide adenine dinucleotide phosphate) oxidase (NOX)-mediated oxidative stress plays a key role in promotion of oxidative injury in the cardiovascular system. The aim of this study is to evaluate the status of NOX in endothelial progenitor cells (EPCs) of hyperlipidemic patients and to assess the correlation between NOX activity and the functions EPCs.
Methods
A total of 30 hyperlipidemic patients were enrolled for this study and 30 age-matched volunteers with normal level of plasma lipids served as controls. After the circulating EPCs were isolated, the EPC functions (migration, adhesion and tube formation) were evaluated and the status of NOX (expression and activity) was examined.
Results
Compared to the controls, hyperlipidemic patients showed an increase in plasma lipids and a reduction in EPC functions including the attenuated abilities in adhesion, migration and tube formation, concomitant with an increase in NOX expression (NOX2 and NOX4), NOX activity, and reactive oxygen species production. The data analysis showed negative correlations between NOX activity and EPC functions.
Conclusions
There is a positive correlation between the NOX-mediated oxidative stress and the dysfunctions of circulating EPCs in hyperlipidemic patients, and suppression of NOX might offer a novel strategy to improve EPCs functions in hyperlipidemia.
INTRODUCTION
Hyperlipidemia, including hypercholesterolemia and hypertriglyceridemia, is considered a major risk factor for atherosclerosis and atherosclerosis-associated diseases such as coronary heart disease, stroke, and hypertension. Accumulating evidence suggests that increased plasma lipids, such as low density lipoprotein (LDL) and oxidized low density lipoprotein (ox-LDL) in particular, can result in vascular endothelial dysfunction and attenuate the repairment of lipoprotein-mediated endothelial injury via several mechanisms such as oxidative stress and inflammatory reaction [1-3]. Endothelial progenitor cells (EPCs), a heterogeneous cell population, are capable of differentiating into the endothelial lineage. EPCs are primarily derived from bone marrow (BM), but they can also be isolated from umbilical cord or peripheral blood. EPCs are thought to function as a backup system to repair endothelial injury so as to maintain vascular endothelial homeostasis [4-6]. Recent studies show that circulating EPCs contribute to the repairmen of endothelial injury in hypertension associated with hypercholesterolemia [7]. There is evidence that reduced numbers and impaired functional capacity of EPCs are found in cell culture with ox-LDL or in patients with hypercholesterolemia [8,9]. Under such conditions, the functions of EPCs in maintaining endothelial integrity and vascular homeostasis are compromised. It is not known, however, what are the potential mechanisms responsible for dysfunction of EPCs under the condition of hyperlipidemia.
Oxidative stress occurs when there is an increased production of reactive oxygen species (ROS) and/or a significant decrease in antioxidant enzyme activity. It has been shown that ROS plays critical roles in regulating stem and progenitor cell bioactivity and that oxidative stress not only reduces EPCs numbers but also impairs EPCs functions (such as migration, adhesion and neovascularization) in various pathological situations [10-12]. Of the multiple sources of ROS in the blood vessel, nicotinamide adenine dinucleotide phosphate (NADPH) oxidase (NOX) is the primary one [5,13]. There are reports that over production of NOX-derived ROS not only hurts the endothelial cells but also causes EPCs dysfunctions [5,14,15]. In hypercholesterolemic mice, administration of NOX inhibitor (apocynin) reduces ROS production and improves the functions of endothelial cells [11,16]. The production of ROS is dramatically increased in patients with hyperlipidemia. It is unclear, however, whether NOX is also activated in EPCs from hyperlipidemic patients and whether the NOX-derived ROS is related to the functional impairment of EPCs.
The aims of this study were to explore the status of NOX in EPCs from hyperlipidemic patients and its association with EPCs functions. In this study, by collecting the EPCs from peripheral blood of the hyperlipidemic patients and the controls, we evaluated the correlation between NOX activity and EPCs functions.
METHODS
Study subjects
Patients with hyperlipidemia (n = 30) and healthy volunteers (n = 30) from Xiangya Hospital (Changsha, China) were enrolled for this study. All patients were newly diagnosed with hyperlipidemia based on the results of total cholesterol (TC) and/or total triglycerides (TG) examinations. Exclusion criteria included cardiomyopathy, congenital heart disease, liver or renal diseases, bleeding disorders, and malignant diseases. Age- and sex-matched controls were selected from community-based inhabitants, free of any hyperlipidemia following the same exclusion criteria as mentioned above. The relevant characteristics of hyperlipidemic patients and controls are summarized in Table 1.
Isolation and characterization of EPCs
Peripheral venous blood samples (15 mL) from the enrolled patients or controls were collected in a sterile tube containing the anticoagulant heparin for plasma or EPCs isolation. EPCs were isolated and cultured according to instructions provided by commercial kits. Briefly, EPCs were obtained by density gradient centrifugation with Ficoll-Paque PREMIUM (GE-Healthcare, Freiburg, Germany). After washing, 107 cells were seeded on culture plates coated with human fibronectin (Millipore, Temecula, CA, USA) and maintained in endothelial basal medium-2 (Lonza, Walkersville, MD, USA) containing endothelial growth medium-2 (EGM-2) SingleQuots (Lonza). At the 7th day of culture, EPCs were identified by immunofluorescence staining to detect surface markers and dual binding characteristics of acetylated LDL incorporation and lectin.
Measurement of plasma lipids
For the measurement of the levels of total TC, TG, LDL and high density lipoprotein in plasma, commercial diagnostic kits were used and the measurements were carried out according to the instructions provided by the manufacturer (Jiancheng Institute of Biotechnology, Nanjing, China). The levels of ox-LDL in plasma were assayed using a commercial ELISA (enzyme-linked immunosorbent assay) kit (R&D Systems, Minneapolis, MN, USA).
Immunofluorescence staining
EPCs were verified by immunofluorescence staining with primary antibody against CD34 (Santa Cruz Biotechnology, Santa Cruz, CA, USA), fetal liver kinase 1 (Flk-1, Abcam, Cambridge, MA, USA), CD144 (Santa Cruz Biotechnology), CD31 (Abcam), endothelial nitric oxide synthase (eNOS, Life Science, Shanghai, China), followed by a secondary antibody of goat anti-rabbit Cy3-conjugated immunoglobulin G (IgG, Beyotime, Jiangsu, China) or goat anti-mouse DyLight 488-conjugated IgG (Beyotime). The cell nuclei were labeled with 4’,6-diamidino-2-phenylindole (DAPI, Beyotime). Images were taken under a fluorescence microscope (Olympus IX71, Olympus, Tokyo, Japan). For the negative control, PBS was utilized to replace the primary antibodies.
Functional analysis of EPCs
The functions of EPCs were assessed through evaluating the abilities of migration, adhesion and tube formation. For migration assay, 1 × 105 EPCs were put in the upper chamber of a 24-well Transwell (Corning, NY, USA), and 600 μL EGM-2 media containing 50 ng/mL of vascular endothelial growth factor were placed to the lower compartment of the chamber. After 6 hours incubation at 37°C, media in the upper and lower chamber were aspirated and EPCs on the top membrane were gently wiped off with a cotton swab. The Transwell membrane was washed with PBS and then fixed in 4% paraformaldehyde for 15 minutes at room temperature. For quantitation, 0.1% crystal violet solution (Beyotime) was used to stain cells, and cells migrating into the lower chamber were counted manually in ten random fields under ×200 magnification.
For adhesion assay, 5 × 104 EPCs were seeded in 12-well plates coated with 10 μg/mL human plasma fibronectin (Millipore). After 30 minutes incubation, plates were washed three times with PBS to remove non-adherent cells, and then incubated in 4% paraformaldehyde. Cells were stained with DAPI (Beyotime) and counted in five random fields under ×100 magnification.
For tube formation assay, Matrigel basement membrane matrix (ECMatriX, BD Biosciences, Franklin Lakes, NJ, USA) was thawed at 4°C overnight. Then 60 μL matrigel was added in a 96-well plate for 1 hour at 37°C. Ten thousand EPCs were added on the top of the solidified matrix containing EGM-2. After 16 hours incubation, tube morphology was imaged and 10 random fields were counted for the tube numbers under ×40 magnification.
Measurement of ROS production in EPCs
Intracellular ROS levels were measured based on the fluorescent signal of 2’,7’-dichlorodihydrofluorescein diacetate (DCFH-DA), a cell-permeable ROS indicator (Beyotime). Non-fluorescent DCFH-DA is oxidized to fluorescent DCF by intracellular ROS once the acetate groups are removed. Briefly, EPCs were washed with PBS and then incubated with 10 μM DCFH-DA at 37°C for 20 minutes. Then, the ROS-mediated fluorescence was monitored under a fluorescent microscope with excitation at 502 nm and emission at 523 nm. Arbitrary fluorescent units were normalized to the control and presented as fold changes.
Measurement of H2O2 production in EPCs
To measure the H2O2 generation in EPCs, mixtures of 50 μL of EPCs lysate and 100 μL of work solution (0.25 mM ammonium ferrous II sulfate, 100 mM sorbitol, 25 mM H2SO4, and 125 mM xylenol orange) were incubated at room temperature for 20 minutes. The change of absorbance at 560 nm was observed, and the level of H2O2 was computed according to a standard curve made from the standard solutions offered by the supplier (Beyotime).
Measurement of NOX activity
The NOX activities were measured by analyzing the NADPH-dependent superoxide dismutase (SOD)-inhibited cytochrome c reduction (Genmed, Shanghai, China). Briefly, the reaction buffer (900 μL) with the NOX substrate (NADPH) and the oxidized cytochrome c was incubated in a quartz cuvette at 30°C for 3 minutes, and then an aliquot (100 μL) of the supernatant from EPCs lysate was incubated with the reaction mixture at 30°C for 15 minutes. The change of absorbance at 550 nm was detected in a spectrophotometer. SOD-inhibited cytochrome c reduction in buffer blank was monitored and subtracted from each sample. The NOX activity was evaluated as SOD-inhibited cytochrome c reduction and expressed as O2– in nmol/mg/min.
Real-time polymerase chain reaction
Real-time polymerase chain reaction (PCR) was performed to determine mRNA levels of NOX2 and NOX4 in EPCs, and β-actin served as a loading control. The primers for NOX2, NOX4, and β-actin were listed in Table 2. Real-time PCR was conducted following standard protocols. Briefly, 2 μg of total RNA from each sample was subjected to reverse transcription reaction by Reverse Transcription System (Takara, Kyoto, Japan) to obtain the cDNAs. Then, 10 μL of reaction mixtures containing 5 μL SYBR Master mix, 2 μL cDNA template, 2.4 μL H2O, 0.20 μL ROX, and 0.20 μL of each primer were amplified following the procedures: denaturing at 95°C for 10 minutes and 45 cycles of the amplification steps (denaturation at 95°C for 15 seconds, annealing and extension at 60°C for 1 minute). Data analysis was carried out by comparative cycle threshold method using 7300 System SDS Software (Applied Biosystems, Foster City, CA, USA). Results were expressed as the ratio of NOX2 or NOX4 mRNA to β-actin mRNA.
Western blot analysis
EPCs were homogenized in ice-cool lysis buffer, sonicated for 1 minute, and then centrifuged at 15, 000 ×g for 15 minutes. The protein concentration in supernatants was determined by a BCA Protein Assay kit (Beyotime). Samples containing ∼40 μg of protein were loaded to 10% SDS-PAGE (sodium dodecyl sulfate polyacrylamide gel electrophoresis) gel and then transferred to polyvinylidene fluoride membranes. The membranes were incubated with primary antibodies against NOX2, or NOX4, followed by horseradish peroxidase (HRP)-conjugated secondary antibodies. The signals of bands were examined using Luminata Crescendo Western HRP substrate through Molecular Imager ChemiDoc XRS System (Bio-Rad, Philadelphia, PA, USA). The densitometric quantification was performed with Image J 1.43 (NIH, Bethesda, MD, USA; https://imagej.nih.gov/ij/). Antibody against β-actin (Beyotime) was used as loading control.
Ethics
This study has been performed in accordance with the Declaration of Helsinki (2013) of the World Medical Association, and was approved by the local Ethics Committee at Xiangya Hospital of Central South University (Changsha, China). Written informed consent was obtained from all individuals at the time of enrollment. All investigators were qualified to undertake this study.
Statistics
The statistical analysis was conducted using the SPSS version 20.0 (IBM Corp., Armonk, NY, USA). Data were presented as mean ± standard error of the mean (SEM). Differences in measured values between two groups were assessed by Student t test. For categorical variables, the chi-square test was used. The correlations between functions of EPCs and NOX activity were calculated using Pearson correlation coefficient. Differences were considered as significant when p < 0.05.
RESULTS
Phenotypic characterization of human EPCs
The isolated mononuclear cells from human peripheral blood samples were cultured for 7 days. At the 7th day, EPCs were identified as the attached cells, showing functions of acetylated LDL uptake (incorporation) and lectin binding (Fig. 1A), as well as expressing the stem cell marker (CD34) and endothelial cell markers (eNOS, CD144 Flk-1, and CD31) (Fig. 1B).
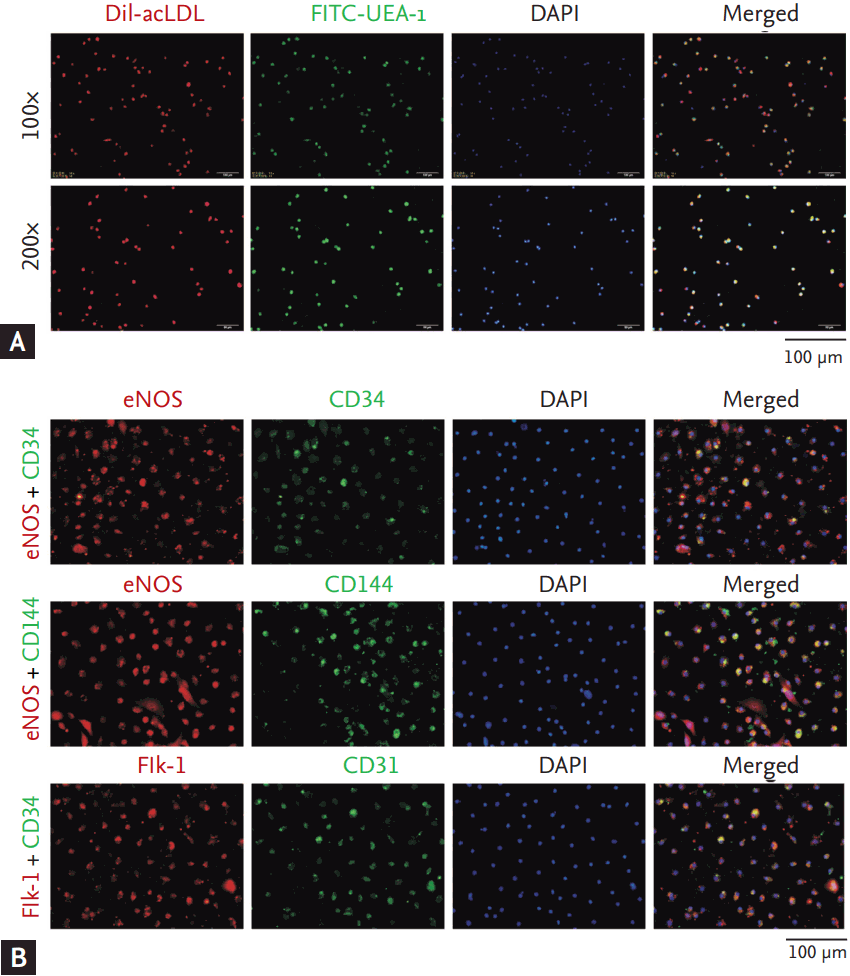
Characterization of human endothelial progenitor cells (EPCs) from peripheral blood. (A) Three-color fluorescent imaging shows Dil-acetylated low density lipoprotein (Dil-acLDL) incorporation (red) and FITC lectin (FITC-UEA-1) binding (green) by EPCs. Nuclei appear dark blue (DAPI). (B) Immunof luorescence staining indicates the expression of CD34 (the stem cell marker), Flk-1, CD31, CD144, and eNOS (the endothelial cell markers) in EPCs. Nuclei were counterstained with DAPI (blue).
Dysfunction of circulating EPCs in hyperlipidemic patients
The functions of EPCs including the abilities of migration, adhesion, and tube formation were evaluated. Compared to the controls, EPCs migration was dramatically reduced in the hyperlipidemia patients (Fig. 2A), concomitant with decreases in adhesive EPCs numbers (Fig. 2B) and tube numbers (Fig. 2C).
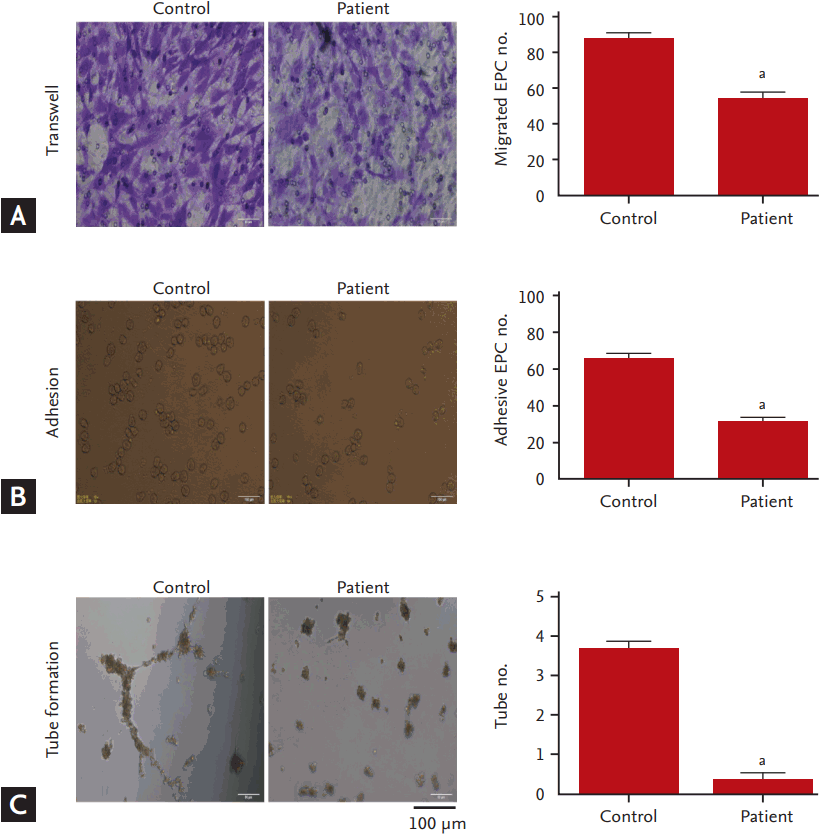
Dysfunctions of the circulating endothelial progenitor cells (EPCs) in hyperlipidemic patients. (A) Left panel, representative images for EPCs migration (Transwell) assay; right panel, the migrated EPC numbers. (B) Left panel, representative images for EPCs adhesion assay; right panel, the adhesive EPC numbers. (C) Left panel, representative images for EPCs tube formation assay; right panel, the tube numbers. All values are presented as mean ± SEM (7 individual samples per group for migration, adhesion and tube formation analysis). ap < 0.01 vs. control.
Up-regulation of NOX in EPCs from hyperlipidemic patients
To investigate whether NOX, a major source of ROS in cardiovascular system, involved in the dysfunction of EPCs in hyperlipidemic patients, we measured the expression of NOX (NOX2 and NOX4) and analyzed the correlation between NOX activity and EPC functions. Compared to the controls, mRNA and protein levels of NOX2 and NOX4 in the EPCs of the hyperlipidemic patients were significantly up-regulated (Fig. 3). Consistently, NOX activity was dramatically increased concomitant with an elevation in plasma levels of H2O2 and the intracellular ROS levels (Fig. 4). The correlation analysis showed that NOX activity in EPCs was inversely correlated with the EPC functions including the abilities of migration and adhesion (Fig. 5).
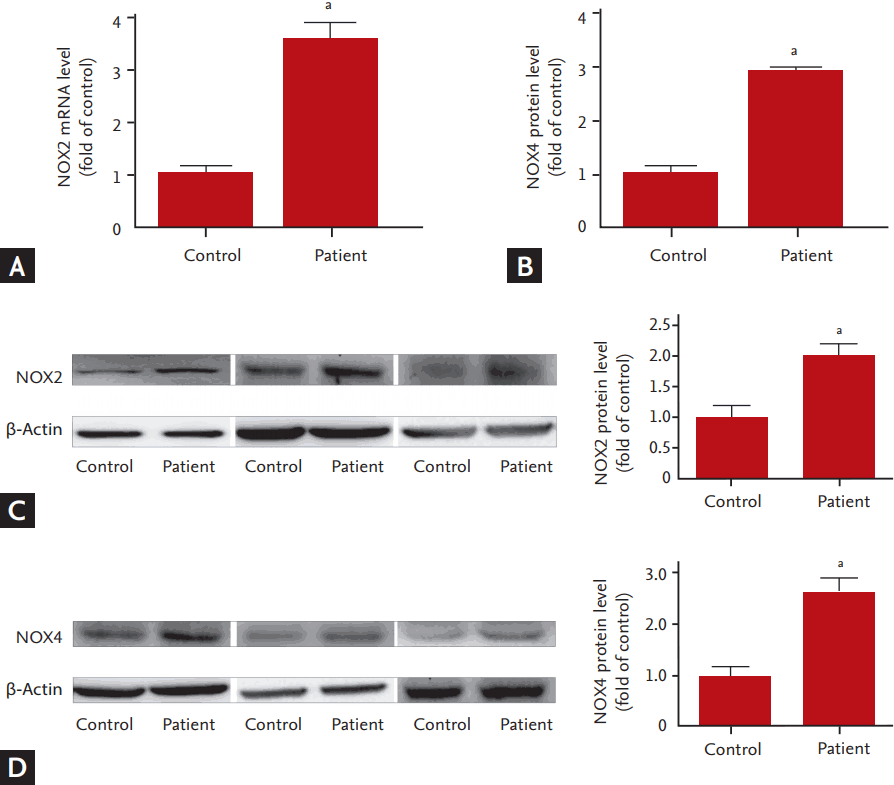
Up-regulation of NOX2 and NOX4 in endothelial progenitor cells (EPCs) of hyperlipidemic patients. (A, B) NOX2 or NOX4 mRNA expression. (C, D) NOX2 or NOX4 protein expression. Left, representative images of Western blot results for NOX2 or NOX4 and β-actin; right, relative changes in optical density for NOX2 or NOX4. All values are presented as mean ± SEM (6 individual samples per group for mRNA and protein analysis, respectively). NOX, NADPH oxidase. ap < 0.01 vs. control.
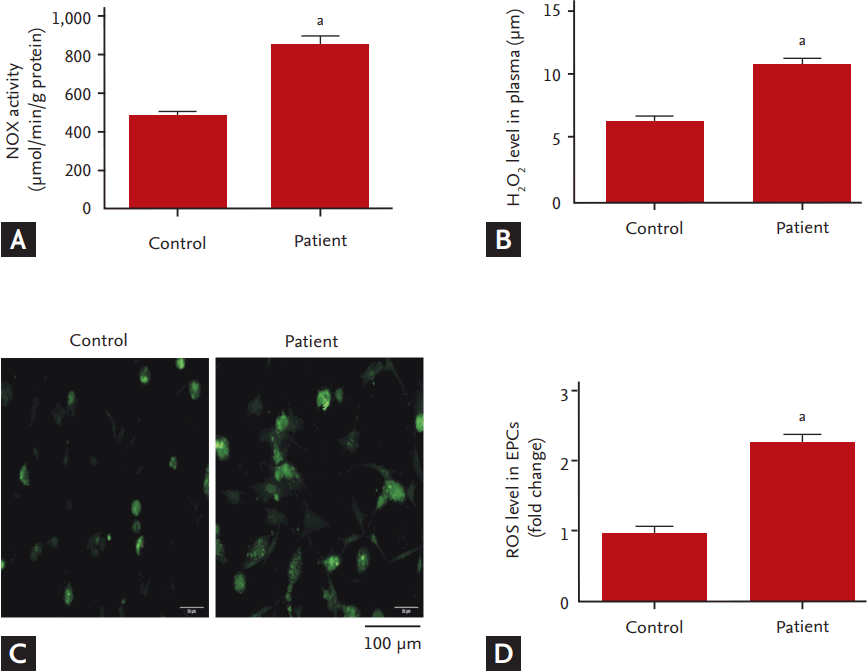
Increase of NADPH oxidase (NOX) activity and reactive oxygen species (ROS) production in endothelial progenitor cells (EPCs) of hyperlipidemic patients. (A) NOX activity in EPCs. (B) H2O2 levels in plasma. (C) Representative image of fluorescent signal of 2’,7’-dichlorodihydrof luorescein diacetate (DCFHDA) for total ROS in EPCs. (D) Statistic value for f luorescent density. All values are presented as mean ± SEM (7 individual samples per group for NOX activity and ROS level analysis). ap < 0.01 vs. control.
DISCUSSION
In the present study, we investigated the correlation between NOX and circulating EPCs functions in patients with hyperlipidemia. Our results showed that EPCs functions (migration, adhesion, and tube formation) were impaired in the patients with hyperlipidemia, accompanied by up-regulated NOX (NOX2 and NOX4) expressions, increased NOX activity, and NOX-derived products (H2O2). The NOX activity was inversely correlated with the EPC functions (the abilities of migration and adhesion). To the best of our knowledge, this is the first study to provide evidence that NOX-mediatedoxidative stress is positively related to the dysfunctions of circulating EPCs in patients with hyperlipidemia.
Vascular endothelium plays a key role in regulation of vascular tone, structure, growth, and homeostasis, and thus protects the vessels from inflammation, immune reaction and thrombosis [17,18]. Multiple stimuli (such as hyperlipidemia and hyperglycemia) can damage the endothelium, which triggers the concomitant invasion of macrophages and lipid depositions, a critical step in atherosclerosis development [19]. Therefore, prompt recovery of the injured endothelium is important for preventing the progression of atherosclerotic vascular disease in patients at risk. EPCs, a cluster of precursor cells that express some cell surface markers of stem cells and endothelial cells, have been reported to contribute to the restoration of the endothelial monolayer [20]. EPCs can be mobilized from the BM, by various stimuli, into the peripheral blood and contribute to the neo-angiogenic process or to the repair of the damaged endothelial cells [17]. There is evidence that the EPCs functions are impaired in hyperlipidemic patients, which may contribute to, at least partly, the endothelial dysfunction in such patients. Consistent with these reports, we have also found the EPCs functions (migration, adhesion, and tube formation) were attenuated in the hyperlipidemic patients. However, the underlying mechanisms are not fully elucidated.
Accumulating evidence demonstrates that oxidative stress is involved in hyperlipidemia-induced endothelial injury and dysfunction. The levels of ROS are significantly elevated in hyperlipidemic patients. It is well known that ROS are produced from several differentially localized and expressed enzyme systems including NOX, xanthine oxidase and cytochrome p450. Among them, NOX is a major source of ROS by transferring electrons from NADPH to oxygen to generate superoxide anion, which in turn dismutates into H2O2 either spontaneously or catalyzed by SOD [21,22]. Reports from other labs and ours repeatedly demonstrated that NOX-derived ROS accounts for cellular oxidative injury under multiple pathological conditions such as ischemia, hyperglycemia and hypertension [23-28]. It is reasonable to predict that NOX-derived ROS may also involve in EPCs dysfunctions in hyperlipidemic patients. Our results clearly showed that the NOX activity in EPCs was dramatically elevated concomitant with an increase in ROS (H2O2) production. To evaluate the correlation between NOX activity and EPCs functions, we performed the correlation analysis. The results showed that NOX activity in EPCs was inversely correlated with EPCs functions (migration and adhesion). These results suggested that NOX-derived ROS might be associated with EPCs dysfunctions in hyperlipidemic patients.
NOX family comprises seven members, named NOX1 to NOX5, dual oxidase (Duox)-1 and Duox-2. Among these isoforms, NOX1, NOX2 (also named as gp91phox) and NOX4 are major isoforms expressed in cardiovascular systems; NOX3 is highly expressed in the cochlea; NOX5 mainly exists in lymphoid tissue and testis while Duox-1 and Duox-2 are predominantly expressed in thyroid epithelial cells [5]. Recently, we have demonstrated that NOX2 and NOX4 expressions in EPCs from pulmonary hypertensive rats are dramatically up-regulated [29]. There is no significant change in NOX1 expression while the NOX3 mRNA expression is not detectable. We thus focused on NOX2 and NOX4 in the present study. Similarly, NOX2 and NOX4 expressions were dramatically up-regulated in EPCs from the hyperlipidemic patients, indicating that selective inhibition of NOX subtypes such as NOX2 and/or NOX4 may provide a strategy to prevent EPCs dysfunctions in patients with hyperlipidemia. However, the mechanisms responsible for the up-regulation of NOX2 and NOX4 in hyperlipidemia need to be further investigated.
The results presented in this study demonstrate, for the first time, that NOX was up-regulated in EPCs from the hyperlipidemic patients, and NOX-derived ROS was positively correlated with the EPCs dysfunctions. Thus, increased NOX might be a novel risk factor for EPCs dysfunctions in hyperlipidemia, and targeting NOX, particularly NOX2 and/or NOX4, may have clinical potential in treating endothelial dysfunction-related diseases.
KEY MESSAGE
1. Hyperlipidemic patients show a reduction in endothelial progenitor cell (EPC) functions (migration, adhesion, and tube formation).
2. NADPH (nicotinamide adenine dinucleotide phosphate) oxidase (NOX) is up-regulated in EPCs from the hyperlipidemic patients.
3. NOX-derived reactive oxygen species is positively correlated with the EPCs dysfunctions.
Notes
No potential conflict of interest relevant to this article was reported.
Acknowledgements
This work was supported by National Natural Science Foundation of China (No.81370250 to Qi-Lin Ma; No. 81573430 to Xiu-Ju Luo; No.81373409 to Jun Peng), Natural Science Foundation of Hunan Province, China (No.13JJ2008 to Jun Peng; No.2015JJ2156 to Luo XJ).